 |
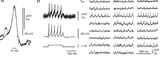
magnified
scene by clicking image
Fig.1 |
Development
of optical recording techniques in our laboratory. Combined optical and microelectrode
recordings of an action potential in a snail neuron (1984), recordings from a
single cell cultured Purkinje cell (1989) and from multiple Purkinje cells in
a cerebellar slice culture (1990). |
|
Introduction
The brain contains many tiny cells, the neurons that talk with each other via
their synaptic connections. Neurons send and receive information and they can
also draw new conclusions from what they have heard from several other neurons,
just to tell this new insight to even others.
Since the pioneering work of Santiago Ramo y Cajal culminating in the neuron
doctrine around the eve of the last century, neuroscientists have discovered and
described the sophisticated communication skills of individual nerve cells. Still,
the understanding of the actions of individual nerve cells such as, the fine tuned
way they exchange and integrate information, is far from being complete. And even,
if we thought we understood the behavior of an isolated neuron, we have to realize
that the actions of ensembles of neurons can only be understood by studying the
context in which their activity occurs. Therefore, we also need to study the "sociology
of neurons", that is the examination of patterns in groups of neurons and the
phenomena evolving from the interaction of neuronal assemblies. The term "sociology"
usually refers to a field of human sciences directed at studying human societies
but many terms and conclusions familiar to sociologists can also be applied to
neuronal interactions at the level of the single brain. As in genuine sociology,
we need an appropriate methodology for experimental observations and a framework
of theoretical analysis to guide our experimental work.
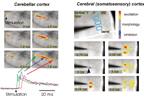
magnified
scene by clicking image
Fig.2 |
Optical
imaging of neuronal activity in cerebellar (left panel) and somato-sensory cortex
(right panel). Electrical stimulation of the surface of the cerebellar cortex
evokes activity in a bundle of parallel fibers followed by postsynaptic responses.
Stimulation below layer IV of the somato sensory cortex evokes activity in two
barrels. |
|
Experimental
Methodology
How can we then observe
the interaction of multiple neurons? Sociologists observe a set of individuals
acting in societies in order to understand the meaning and context of their individual
behavior.
Probably the most straightforward observable reflecting the "group dynamics" of
neurons are the electrical signals (changes in neuronal membrane potentials) associated
with their communication. In a simplistic way, we could say that transmitted messages
of individual neurons are detectable as action potentials and received messages
are mirrored as postsynaptic potentials.
Since many neurons speak to each other at the same time, we need to monitor the
exchange and processing of information from many individual neurons simultaneously.
Given the small size and 3-dimensional packing of neurons, we cannot stick a wire
in each cell in order to record their electrical signals. This technical problem
could be mastered by optical imaging of electrical activity, a technique that
had been proposed many years ago as a less invasive and more promising approach
to investigate the multineuronal representations of information processing in
brain tissue. Fluorescent dyes that stain neuronal membranes and that change their
fluorescence output as a function of the membrane potential have been known for
many years.
My laboratory used such voltage sensitive dyes (VSDs) in a variety of preparations
over the last 15 years (Figs 1 and 2).
More recently, considerable progress has been made in the development of instrumentation
suitable for high speed imaging of electrical activity in mammalian brain slices.
As illustrated in Fig 2, VSD recordings make it possible to visualize the propagation
of action potentials and the subsequent generation of postsynaptic potentials
with high spatio-temporal resolution in cerebral and cerebellar cortices.
While VSDs are still the workhorse for fast optical recordings of membrane potentials,
their application has clear limitations due to low effective sensitivity, potential
toxicity and, most importantly, undefined cellular origin. Protein-based and DNA-encoded
voltage sensors could overcome these limitations. Therefore, we designed and generated
voltage-sensitive fluorescent proteins (VSFPs) consisting of a voltage-sensing
domain of a potassium channel and mutants of green fluorescent protein (GFP).
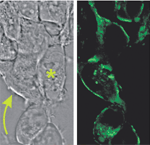
magnified
scene by clicking image
Fig.3 |
Cultured
Cells (HEK 293) expressing a prototypic voltage sensitive fluorescent protein
(VSFP). Left panel shows transmission photomicrograph; right panel shows fluorescence
image. Membranes of VSFP expressing cells (arrow) but not those of non-expressing
cells (*) exhibit bright fluorescence. |
|
In response to a change in transmembrane voltage, the voltage sensor of the potassium
channel imposes a conformational change on the fluorescent protein which in turn
alters the amount of fluorescence output. This optical readout responds at the
millisecond time scale of fast electrical signaling and is sensitive enough to
allow monitoring voltage changes at the single-cell level. VSFPs provide optical
signals only from those cells targeted by the expression construct. Thus, in intact
tissues, VSFPs targeted to specific cell populations will provide a signal-to-noise
ratio superior to the signals obtained with conventional
voltage- sensitive dyes. The next obvious step is to place the construct under
the transcriptional control of promoters driving gene expression in specific populations
of neurons in transgenic animals. We recently demonstrated the feasibility of
obtaining an optical readout of genetically encoded GFP mutants by generating
transgenic mice expressing a pH and Cl--sensitive GFP variant under the control
of a neuron-subtype specific promotor (Fig. 4).
Theoretical
Framework
Any theory on the operation
of the brain has to define the coding scheme (i.e., the language) of neuronal
communication. The language is a set of rules by which the signals (i.e., action
potentials, articulated sounds) carry information. When focusing only on the output
of an individual neuron (i.e., the temporal sequences of action potentials), one
might debate whether it is the mean firing rate that carries the information (rate-coding
hypothesis) or whether it is the precise placement of the spikes in time that
is the significant feature (temporal-coding hypothesis). The importance of temporal
coding becomes clear when considering many interacting neurons. In sociological
terms the point would be: Of course, it is important what and how much an individual
says, but the significance of what is said strongly depends on when (and by whom)
it was said, and who (if anybody at all) listened and responded (positively or
negatively) to what was said. Thus, only the interaction between neurons gives
meaning to the information content. In addition to exchanging information, neurons
also transform information: During the process of neuronal computation, a synthesis
is derived from the communication within a workgroup of neurons, and the resulting
joint response might have behavioral or cognitive consequences. As we would expect
from a team of people, a joint statement has a much richer content than an isolated
opinion.
Recently, oscillations and synchronization of neuronal activity have attracted
much interest. Do these phenomena relate to complex temporal coding schemes or
do they reflect the process of computation? In sociological terms: Does synchronized
activity reflect per se a joint statement of a team of people or does synchronization
of communication channels provide a condition required for reaching a joint statement?
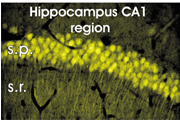
Fig.4 |
Expression
of a pH and Cl--sensitive GFP variant in pyramidal cells in the CA1 region of
the hippocampus. s.p., stratum pyramidale; s.r., stratum radiatum. |
|
Hum an societies
change over time, usually in response to and as an adaptation to external factors.
Much the same happens in the society of neurons. A major part of neuronal computation
can be seen as a process of fitting the external world (as seen by sensory systems)
into internal representations and of adapting and refining of this internal system
based on experience. The latter process obviously is related to learning and memory.
Perspective
During the last years
we have developed and implemented an optical imaging approach that will allow
us, using experimental data, to challenge and develop theoretical concepts concerning
multineuronal activity. We envisage two lines of progress in the near future:
First, we shall find out in more detail how the dynamic interaction of neuronal
activity changes after acquisition of new information. There is general agreement
that, for a period of up to several days, learning occurs at the synaptic level
and can be observed as a change in efficacy of synaptic transmission (how loud
a neuron can speak). For longer-lasting adaptations, we would expect a rearrangement
of information flow via a rearrangement of structural connectivity, as has been
demonstrated in the developing brain. One ambitious goal would be to actually
visualize these learned patterns of neuronal interactions ("memory traces").
A second line of research will contribute to the currently debated hypothesis
that synchronization of neuronal activity is used to combine distributed processing.
Optical imaging techniques might be instrumental in understanding the biophysical
mechanisms and features underlying neuronal oscillations and synchronization.
|
 |
|
|